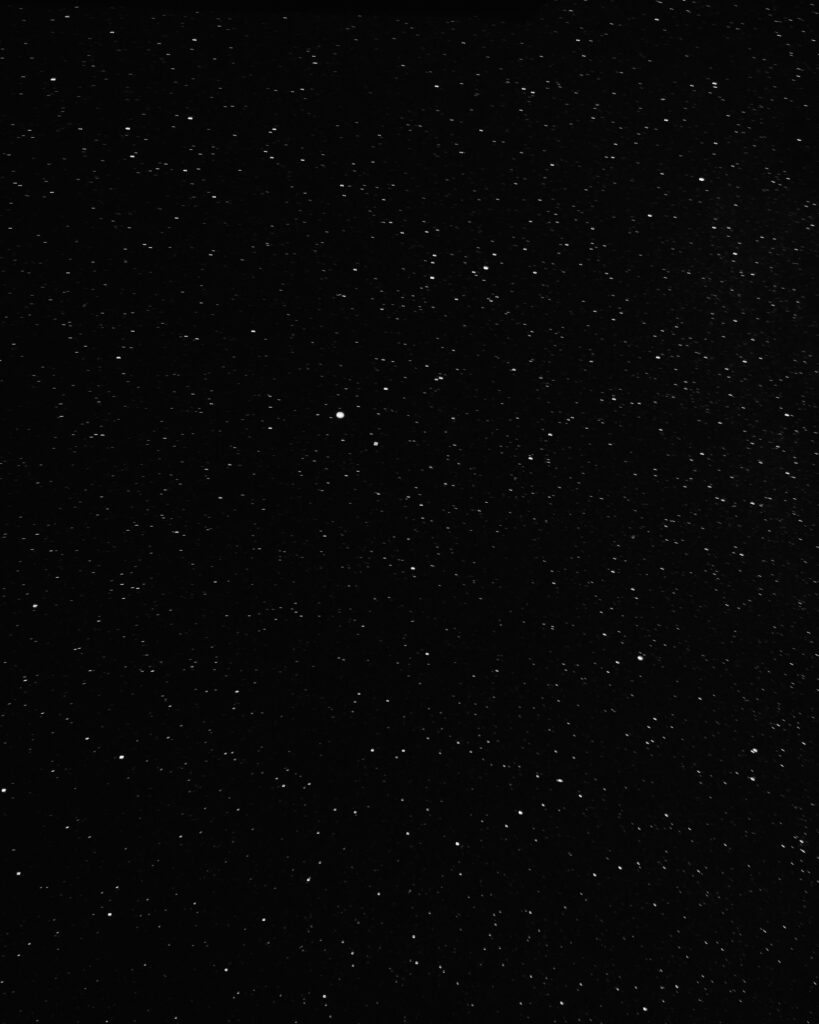
Introduction to Theories in Physics
Scientific theories are crucial conceptual frameworks that help us understand, predict, and explain natural phenomena. In the context of physics, these theories serve as foundational pillars for comprehending the complexities of the universe. A scientific theory is not merely a hypothesis; it is a well-substantiated explanation of some aspect of the natural world, based on a body of evidence that has been repeatedly validated through observation and experiment.
The formulation of theories in physics involves a methodical process that begins with observations. These observations generate questions that require rigorous investigation. Through systematic experimentation and precise measurement, data is gathered, which leads scientists to formulate hypotheses. Mathematical modeling then plays a pivotal role in this process, providing a structured, quantifiable means to frame and test these hypotheses. Theories are continually refined and validated through further experiments, leading to a deepened understanding of the underlying principles governing physical phenomena.
One of the paramount contributions of physics theories is their predictive power. For instance, Newton’s laws of motion not only explain why an apple falls to the ground but also predict the orbital mechanics of celestial bodies. Similarly, quantum mechanics allows for the prediction of particle behavior at the subatomic level with remarkable accuracy. These predictions are essential not just for advancing theoretical knowledge but also for practical applications in technology and industry.
The advancement of scientific knowledge is heavily dependent on these theories. Theories in physics provide the framework necessary for innovation and the development of new technologies. They guide experimental research, inform the design of new experiments, and help interpret experimental results. This cyclical relationship between theory and experimentation drives the progress of science, leading to ever more sophisticated Learn of the universe.
Classical Mechanics: Newton’s Laws of Motion
Classical mechanics, underpinned by Newton’s three laws of motion, remains fundamental to our understanding of the physical world. Introduced by Sir Isaac Newton in his 1687 work, “Principia Mathematica,” these laws offer a comprehensive framework for describing the relationship between motion and the forces affecting it. Newton’s laws have not only laid the groundwork for subsequent advancements in physics but have also had far-reaching effects on engineering and various real-life applications.
Newton’s First Law of Motion, often dubbed the law of inertia, states that an object at rest remains at rest, and an object in motion continues in uniform motion unless acted upon by a net external force. This principle fundamentally shifted the prevailing Aristotelian view and elucidated why objects do not change their state of motion without a cause, such as friction or applied forces.
The Second Law of Motion is articulated through the equation F = ma, where F is the force applied to an object, m is its mass, and a is its acceleration. This law quantitatively describes how forces influence motion, enabling precise calculations essential for modern engineering marvels. For example, understanding this law is crucial for designing automotive safety systems, predicting spacecraft trajectories, and even in the field of biomechanics.
Newton’s Third Law of Motion states that for every action, there is an equal and opposite reaction. This law exemplifies the mutual interactions between objects. A practical manifestation of this law is evident in the propulsion of rockets, where the expulsion of gas exerts a forward thrust.
The importance of Newtonian mechanics extends beyond theoretical constructs; it manifests in countless everyday phenomena. From the simple act of walking, where our feet push against the ground to the energy transfer designs in numerous mechanical systems, these principles are omnipresent. Historically, experiments such as Galileo’s inclined plane paved the way for Newton’s consolidated laws, transforming scientific thought and laying the bedrock for analytical mechanics.
Electromagnetic Theory: Maxwell’s Equations
James Clerk Maxwell’s contributions to physics cannot be overstated, especially his formulation of the electromagnetic theory, solidified in what is now known as Maxwell’s Equations. These four partial differential equations synthesize the principles of electricity and magnetism into a unified framework — laying the groundwork for electromagnetism, a cornerstone of modern physics. Maxwell’s Equations describe how electric charges and currents produce electric and magnetic fields, and how these fields propagate through space.
The four Maxwell’s Equations consist of:
Gauss’s Law: This equation quantifies the relationship between electric charge distribution and the resulting electric field. It essentially says that the electric flux through any closed surface is proportional to the enclosed electric charge.
Gauss’s Law for Magnetism: Unlike electric fields, this law reveals that there are no magnetic monopoles; in other words, magnetic field lines always form closed loops. The net magnetic flux through a closed surface is zero.
Faraday’s Law of Induction: This equation explains how a time-varying magnetic field generates an electric field. It is the principle underlying many forms of electrical power generation, such as in transformers and electric generators.
Ampère’s Law (with Maxwell’s correction): It states that magnetic fields can be generated by electric currents and changing electric fields, combining both effects into a unified framework. This addition by Maxwell corrected earlier theories, allowing the equations to predict the existence of electromagnetic waves.
These equations have had profound implications for both theoretical and applied physics. They predict the existence of electromagnetic waves, including light, radio waves, and microwaves, which propagate through the vacuum at the speed of light. This theoretical foundation has led to numerous technological advancements. For example, the principles of electromagnetism underpin electric power generation and distribution, telecommunications, and wireless technologies.
Experimental validations of Maxwell’s theory are abundant. Heinrich Hertz’s experiments in the late 19th century, which demonstrated the existence of radio waves, directly confirmed Maxwell’s prediction about electromagnetic waves. Furthermore, the development of wireless telegraphy by Guglielmo Marconi and the subsequent innovations in radio and television broadcasting owe much to Maxwell’s theoretical insights.
Theory of Relativity: Special and General
Albert Einstein’s theory of relativity, encompassing both special and general relativity, revolutionized our understanding of physics in the early 20th century. In 1905, Einstein introduced special relativity, which fundamentally altered the conception of space and time. This theory posits that the speed of light is a universal constant, unaffected by the relative motion of the observer. One of the principal outcomes of this theory is time dilation, where time appears to slow down for an object moving at high speeds compared to one at rest. Additionally, length contraction suggests that objects in motion will appear shorter along the direction of travel, compared to those at rest.
In 1915, Einstein presented his theory of general relativity, extending the principles of special relativity to include acceleration and gravity. General relativity proposes that massive objects cause a curvature in spacetime, which we perceive as gravity. An analogy often used is that of a heavy ball placed on a trampoline, causing a dip; lighter objects rolling nearby will move towards the dip, similar to how planets orbit stars. This curvature affects the path of light as well, leading to phenomena such as gravitational lensing, where light bends around massive objects, altering the appearance of distant stars and galaxies. Another key prediction of general relativity is gravitational time dilation, where time passes more slowly in stronger gravitational fields.
Historical experiments have played a pivotal role in validating these theories. The 1919 Eddington expedition famously confirmed general relativity during a solar eclipse by observing the position of stars near the Sun, proving light bends due to gravity. Similarly, the Hafele-Keating experiment in 1971, involving atomic clocks flown around the Earth, confirmed predictions of time dilation by special relativity. These groundbreaking theories are not merely academic; they underpin modern technologies like GPS, which accounts for time dilation effects in its calculations. Furthermore, they have profound implications for our understanding of black holes, the expansion of the universe, and the quest for a unified theory in cosmology.
Quantum Mechanics: Uncertainty and Probability
Quantum mechanics remains one of the most essential yet enigmatic frameworks in modern physics. At its core, the theory delves into the peculiar nature of particles at the atomic and subatomic levels, presenting principles that profoundly differ from the deterministic world of classical mechanics. One of the foundational concepts introduced by quantum mechanics is the wave-particle duality, which posits that particles such as electrons exhibit both wave-like and particle-like properties depending on the experimental context.
A significant breakthrough in quantum theory is the Heisenberg uncertainty principle, articulated by Werner Heisenberg in 1927. This principle asserts that it is fundamentally impossible to simultaneously measure both the exact position and momentum of a particle with infinite precision. The uncertainty principle highlights the intrinsic limitations within quantum systems, challenging the determinism upheld by classical physics. As a result, it reshapes our understanding by suggesting that the very act of observation alters the phenomena being observed.
Furthermore, the probabilistic nature of quantum states marks a departure from classical mechanics. Instead of predicting a single, definitive outcome, quantum mechanics relies on probability distributions to describe the likelihood of finding a particle in a particular state. This is vividly illustrated in the iconic double-slit experiment, where particles such as electrons create an interference pattern when passed through two slits, suggesting wave-like behavior. However, when observed, the particles seem to choose one path, demonstrating particle-like characteristics. This duality underscores the complex and non-intuitive behavior of quantum systems.
Quantum mechanics also has profound applications in modern technology. In the emerging field of quantum computing, principles such as superposition and entanglement enable the development of computers exponentially more powerful than classical ones. Additionally, quantum cryptography leverages quantum properties to create theoretically unbreakable communication protocols, promising heightened security in data transmission.
Overall, quantum mechanics continues to intrigue and challenge physicists, offering a deeper glimpse into the fabric of our universe and laying the groundwork for revolutionary technological advancements.
“`
Modern Theories: String Theory and Quantum Gravity
In recent decades, the quest to unravel the foundational mysteries of the universe has led physicists to develop groundbreaking theories such as string theory and quantum gravity. These modern theories aim to solve enduring puzzles about the fundamental nature of reality, including the elusive goal of unifying quantum mechanics with general relativity.
String theory, in particular, represents a dramatic shift in our understanding of the universe’s building blocks. Unlike traditional theories that describe particles as point-like entities, string theory posits that the most basic elements are one-dimensional “strings” that vibrate at different frequencies. These vibrations manifest as various particles, akin to how different musical notes can be produced by the same violin string. Additionally, string theory introduces higher-dimensional objects known as “branes.” These multi-dimensional membranes further expand on the concept, suggesting that our familiar three-dimensional world might be part of a higher-dimensional framework.
The motivations behind string theory stem from the necessity to reconcile quantum mechanics—successful at describing the behaviors of particles at the smallest scales—with general relativity, which excels at explaining gravitational phenomena on cosmic scales. Traditional physics struggles to merge these two pillars into a coherent framework, especially when confronting extreme conditions such as those in black holes or the big bang.
Quantum gravity seeks to address this unification as well. Researchers in this field strive to formulate a theory that integrates quantum mechanics with the geometric principles of general relativity. Quantum gravity attempts to demystify phenomena that remain unexplained by current physics, such as the quantum behavior of black hole event horizons and the singularity—an infinitely dense point—predicted by the big bang model.
Efforts to develop a comprehensive theory of quantum gravity include various approaches like Loop Quantum Gravity and the ADS/CFT correspondence, each offering unique insights. While a definitive theory remains elusive, these innovative frameworks provide the exciting potential to unveil new dimensions of understanding about our universe, marking significant milestones in our intellectual venture to comprehend the cosmos.